Harmonic Generation and Frequency Multiplication
Figure: Cross Waveguide Harm-
onic Generator
While frequency multiplication can be traced to the dawn of the microwave era [1]. Figure: Cross Waveguide Harmonic Generator shows a drawing of this device. Since it was designed for spectroscopy, broad spectral coverage was emphasized and only back shorts were included for tuning. Not only are these multipliers broadbanded across an entire waveguide input band, but many harmonics were generated simultaneously.
Since linear molecules have series of absorptions in almost, but not exactly, harmonic relation, the multiple harmonic outputs can be separated and observed by scanning the input frequency of the multiplier through the closely spaced subharmonics of the different absorption frequencies. The resultant closely spaced (in source frequency) absorptions are shown in Figure: Harmonic Series of OCS Absorptions [4]. Similar devices, some with matching optimized for narrow spectral intervals and operating in chains of low order multiplication, are the sources of choice for many applications requiring local oscillator power for receivers in remote sensing instruments. Examples of these instruments will be discussed in more detail below.
Because absorption spectroscopy is linear, increased detector sensitivity can be used to compensate for reduced source power. Thus the introduction [5].resulted in a significant advance for THz spectroscopy.
Although painstaking, a large body of work resulted from this approach [8]. Not only was harmonic generation the basis for the initial explorations of the THz spectral region, for many applications it continues to be the technology of choice. In combination with sensitive detector technology, it provides broad spectral coverage, simple frequency control and measurement, high resolution and sensitivity, and overall system reliability and simplicity.
Figure: Harmonic series of OCS absorptions. Shown in this figure are absorptions in the 12th (291 GHz) through 21st harmonics (517 GHz) of a klystron operating near 24.325 GHz. |
Figure: Ramsey Fringes in H2S. Ramsey fringes in the spectrum of H2S obtained with a molecular beam electric resonance system. |
The resolution of most THz spectroscopy is limited by Doppler broadening to a $Q$ of about $10^6$. While this is satisfactory for most applications, higher resolution is often important. As in many Doppler limited applications, molecular beam approaches have been used in the THz both for molecular beam masers [9]. Figure: Ramsey Fringes in H2S shows a spectrum obtained with this device. Another important application of THz spectroscopy has been the study of molecular ions. Not only is their production of fundamental interest, their relative abundance in the interstellar medium has made them of significant astrophysical interest as well. After a demonstration by Woods and his coworkers [12].that the positive column of glow discharges contained observable quantities of molecular ions, a number of workers were attracted to the field.
Modern microfabrication and broadband circuit design have significantly expanded the capabilities of frequency multiplication [13]. The reliability of these devices has broadened the user community as well. The commercial availability of frequency multiplication and related technology from Virginia Diodes, Inc. has contributed as much as anything to scientific and technology advances in the mm/submm.
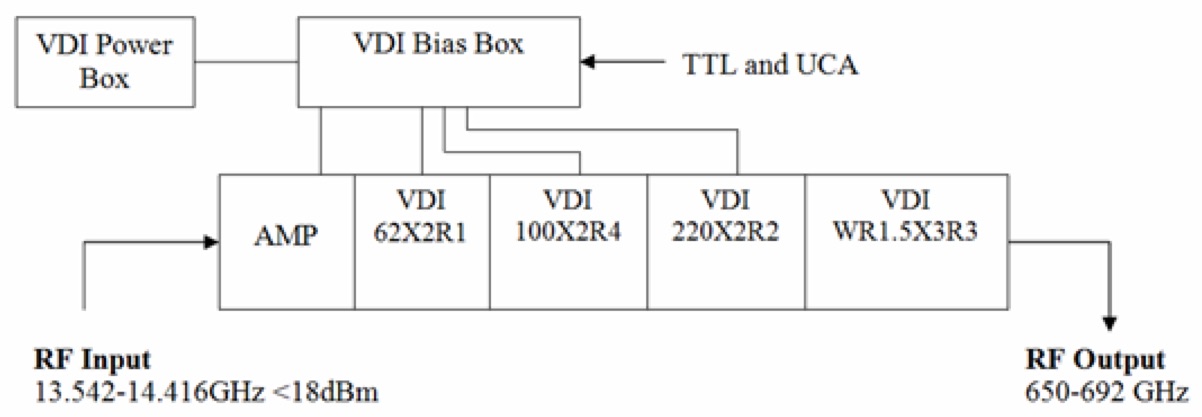
It is noteworthy that it is this approach is the technology of choice for all of the recent major systems, for example ALMA, Herschel, and MLS. However, with the wider frequency ambitions of SOFIA, approaches, such as Quantum Cascade Lasers (QCLs) from the other side of the SMM gap are under active consideration. [Rabanus, 2009 #14511][14].
References
- Transient Nutations in Nuclear Magnetic Resonance Phys. Rev. 76, 1059-1068 (1949). Google Scholar
- One-to-Two Millimeter Wave Spectroscopy. IV. Experimental Methods and Results for OCS, CH3F, and H2O Phys. Rev. 93, 407-412 (1954). Google Scholar
- One to Two Millimeter Wave Spectroscopy. I Phys. Rev. 90, 319-320 (1953). Google Scholar
- Precision Measurements of Millimeter and Submillimeter Wave Spectra Phys. Rev. 111, 209-211 (1958). Google Scholar
- Extension of Microwave Absorption Spectroscopy to 0.37-mm Wavelength Phys. Rev. Lett. 25, 1397-1399 (1970). Google Scholar
- Continuously Tunable Coherent Spectroscopy for the 0.1- to 1.0-THz Region Appl. Phys. Lett. 42, 309-310 (1983). Google Scholar
- Indium Antimonide Submillimeter Photoconductive Detectors Appl. Opt. 4, 649-657 (1990). Google Scholar
- Microwave Spectroscopy in the Region of 4-0.4 Millimeters Pure Appl. Chem. 11, 403-434 (1965). Google Scholar
- Molecular Beam Maser for the Shorter-Millimeter-Wave Region: Spectral Constants of HCN and DCN Phys. Rev. 187, 58-65 (1969). Google Scholar
- Extension of High-Resolution Beam Maser Spectroscopy into the Submillimeter Wave Region Can. J. Phys. 55, 1115-1123 (1977). Google Scholar
- Millimeter Electric Resonance Spectroscopy Proc. IEEE 54, 506-513 (1966). Google Scholar
- Laboratory Microwave Spectrum of HCO+ Phys. Rev. Lett. 35, 1269-1272 (1975). Google Scholar
- Opening the Terahertz Window with Integrated Diode Circuits IEEE J. Solid-State Circuits 40, 2104-2110 (2005). Google Scholar
- Tunable Emission in the THz Quantum Cascade Lasers IEEE Trans. Terahertz Sci. Technol. 1, 76-84 (2011). Google Scholar
- Submillimeter-Wave Spectroscopy of Thioformaldehyde, H2CS, in its Ground State OSU International Symposium on Molecular Spectroscopy 62, 173 (2007). Google Scholar